Understanding Symmetry in Physics: Simplified Science Breakdown
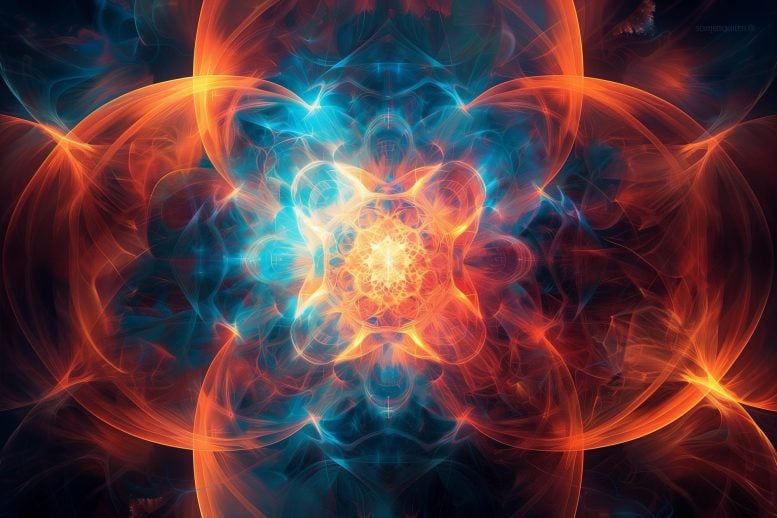
Symmetry in physics explores particle behavior under spatial, temporal, and quantum number reversals, involving both discrete and continuous changes. It underpins the consistency of natural laws and experiment reproducibility, heavily influencing modern physics theories like supersymmetry and potentially unveiling new particles and forces. Credit: SciTechDaily.com
In physics, symmetry refers to how particles behave when space, time, or quantum numbers are reversed. We’re used to seeing simple types of symmetry in everyday life. For example, a human face is very nearly symmetrical when reflected left to right. But a face is completely asymmetric when reflected top to bottom—the top half of a face doesn’t look the same as the bottom. Small changes can break symmetry. For example, a face with a mole on one cheek would break left right symmetry.
Symmetry in physics is about more than just appearance and form. This is in contrast to symmetry in biology, which often refers to symmetry when discussing how organisms look and how their bodies are arranged.
Symmetry in physics can refer to the laws of nature being immune to changes in the identity or properties of certain elementary particles. Symmetry can also mean changes in what may seem to be abstract mathematical descriptions of nature.
A clock face is an example of parity transformation. The clock face’s appearance and behavior if the coordinate system is reversed is a test of symmetry. This image shows how the clock looks and works if it is reflected in a mirror. Credit: Nathan Clarke, Department of Energy Office of Science
Symmetry often refers instead to how nature behaves when particles are swapped with their anti-particles (called “charge conjugation”), when coordinates are reversed as in a mirror (called “parity inversion”), or when time is reversed (running the “movie” backward). Physicists call these three symmetries C (for charge), P (for parity), and T (for time). So-called CPT symmetry is discrete—it happens in steps.
Physicists contrast discrete symmetry with continuous symmetry, where there are no steps between changes in symmetry. One example is rotational symmetry such as turning a circle. Unlike the case of rotating a square, there are no points where rotating a circle breaks the symmetry.
For scientists, translational symmetry in time and space is especially important. Translational symmetry means the laws of nature measured by one person at one time and in one place will not change if measured by another person at another time and another place. Without this symmetry, the physical sciences and the universal laws of nature wouldn’t work. In other words, symmetry in time and space is what makes experiments reproducible and science possible.
Understanding symmetries and broken symmetries is important for understanding the physical properties of matter and our universe.
Many of the advances in physics over the past several hundred years have been based on an increasing recognition of the importance of symmetry in generating theories of nature. If scientists can correctly deduce which symmetries are valid, they can learn more about what kinds of things are possible in nature, especially with subatomic particles. Many theories that seek to improve upon the standard model of particle physics rely on symmetry as a guiding principle. For example, supersymmetry theory proposes that all the particles in the Standard Model have a symmetric partner. Alternately, if scientists observe a symmetry being broken when they didn’t expect, that finding may point to possible new ways for nature to behave. That can include new particles and new forces.
The search for the fundamental symmetries of nature is such a deep and broad question that it engages physicists with different expertise from four of the programs in the Department of Energy Office of Science.
These programs include Basic Energy Sciences (BES), High Energy Physics (HEP), Nuclear Physics (NP), and Advanced Scientific Computing Research (ASCR).
BES supports techniques to synthesize and characterize novel materials at the atomic level. These materials often exhibit broken symmetries leading to deeper scientific understanding and novel functionality with potential impact for DOE’s energy mission.
NP supports physicists who employ advanced techniques to search for broken symmetries for neutrons, protons, and various nuclear isotopes. NP also supports efforts to search for a yet to be observed nuclear decay known as neutrino-less double beta decay. Observation of this decay would show matter being created in the laboratory without any counterbalancing antimatter, a broken symmetry that is essential to understanding why the universe has enough matter in it for us to exist.
HEP supports physicists searching for ways that symmetry can be violated by using the Deep Underground Neutrino Experiment, the Large Hadron Collider, and other experiments. Meanwhile, ASCR supports the computer theory, hardware, and software scientists needed to conduct and interpret such data-rich experiments.